Dense orbital meshes of internet routers, gateways to manned moon bases, observing massive black hole mergers from space. Fantastic developments are well on the way in our space programmes, which have also become hugely diverse. They range from purely commercial ventures, through (inter-)governmental applications for society, to manned and science missions. In this article I’ll take a quick tour through some of these and highlight some interesting radiation issues. The intention of the article is to throw ideas out and stimulate thought and discussion. So if you don’t agree with what I write or want to add points, feel free via Linkedin. In developing space systems, we have to overcome many difficult engineering hurdles. Just Google “esa science red books” and see great examples of early system engineering studies, addressing the likes of propulsion, thermal control, communications, spacecraft control, structure, and electrical power. The RADNEXT community brings a collection of facilities and skills to address the equally important, but also fascinating, space radiation effects domain. Concerns about radiation effects are quickly growing in interesting new directions that need new thinking and tools in order to address them. Space agencies, European and national, are developing ever more ambitious space missions doing science, observing Earth’s environment and for human and robotic space exploration. At the same time, commercial and public exploitation of space is radically evolving our “electronic infrastructure” such as communications and navigation. In terms of space radiation, these developments pull in different directions and in this article I’ll discuss the some of the needs and interesting problems.
The Competition for the Internet High Ground
SpaceX’s Starlink constellation is in the news a lot these days. As in similar concepts (see the non-exhaustive table below) they essentially aim to put a large internet routing infrastructure in space to reach the large part of the Earth’s population - billions of people - that have no, or poor, internet access. The EU are also funding studies of a constellation – a “secure space-based connectivity system” [1] . The business models rely on being able to provide end users with an affordable service with easy-to-use hardware. The consequence is that cost of the space infrastructure - the “mega-constellation”- must be low and individual satellite costs must be very low. But will the drive to reduce costs lead to lower satellite reliability? And why does that matter? The problem is space debris. While satellites typically have a capability to de-orbit or relocate to an unused orbit, that depends on retaining reliable operation of the de-/re-orbit system at the end of life, unless they’re in a low enough orbit where atmospheric drag can remove them (below ~500km). A population of defunct satellites in orbits with poor drag “cleaning” can lead to a serious and potentially growing debris problem [2] . Addressing reliability inevitably leads one to radiation hardness assurance and clearly these commercial constellations will use as much heritage, standard units, and “safe” COTS as possible. Radiation testing and modelling will need to be there to play key roles in validating designs, and screening components or complete units.
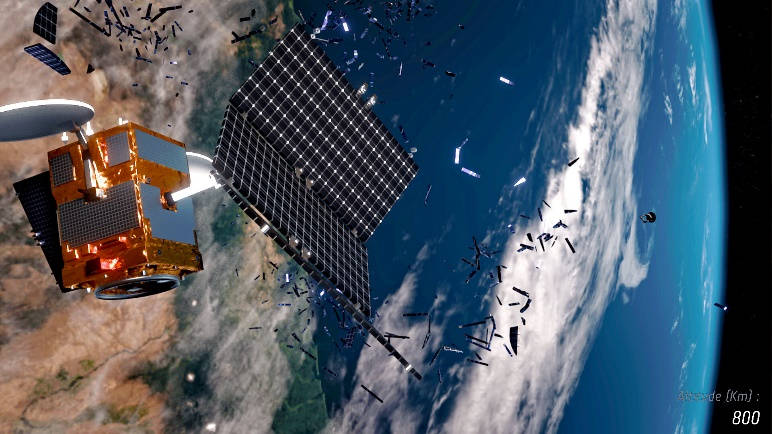
Figure 1: Space debris concerns will inevitably increase with deployment of mega-constellations, Credit: ESA (CC BY-SA 3.0 IGO)
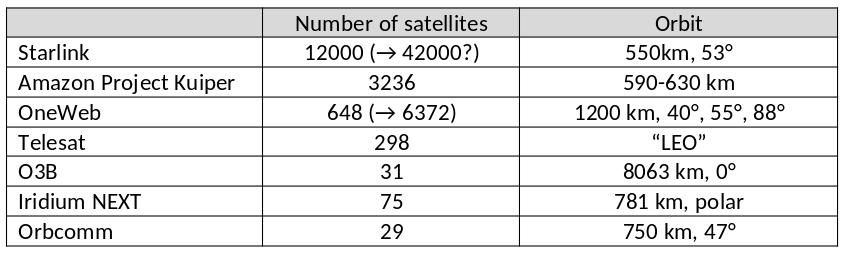
What we think of as the more traditional space telecommunications sector, that being relatively large geostationary (GEO) satellites, is also developing significantly, complementing constellations in other orbits. Competition is not just about numbers of satellites and orbits but also about power, performance and on-board processing. The “big beasts” in GEO these days have greatly increased on-board processing and other capabilities compared to a couple of decades ago. This again raises radiation hardness assurance concerns but another trend leads to additional space environment problems. That is “electric orbit raising” (EOR). The traditional way to get a satellite into GEO is for the launcher to place the satellite in a transfer orbit. The final orbit is then achieved very soon thereafter with the satellite’s on-board apogee boost motor (ABM). Having to carry an ABM means that part of the mass budget that could otherwise be used for profitable payloads is consumed. In space mass equals money. Electric propulsion (EP) is very much lighter by comparison, but the instantaneous thrust is much lower. The orbit raising can still be achieved by continuous or frequent EP operation. The next generation of the European Galileo navigation system, at around 23000 km altitude and 56° inclination, will use a similar scheme.
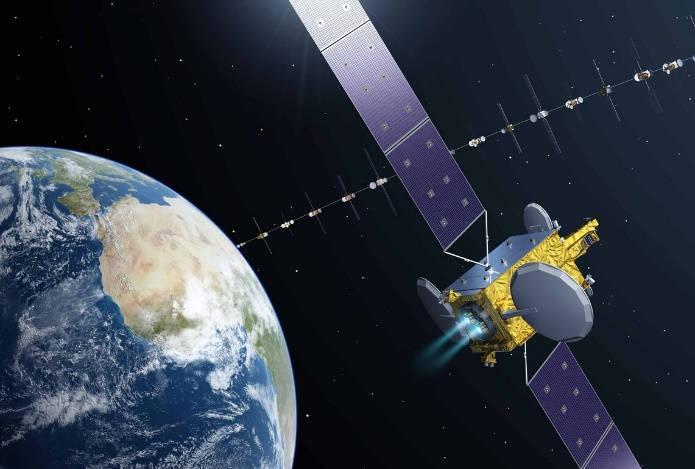
Figure 2: Electric orbit raising: the slow passage through the radiation belts has to be carefully managed, Credit: ESA
The radiation concern comes from the fact that the spacecraft can spend weeks or months slowly transiting through the radiation belts until it reaches a more benign GEO environment (see Figure 3, from [3] ). This can result in degradation of solar cells, single event effects in the proton radiation belt and generally add to the radiation burden of the mission – at the start of the mission. In addition, whereas for long duration GEO missions it is appropriate to work out the radiation budget using knowledge of the long-term average environment, during EOR one must remember that the environment can vary dramatically because of solar-geomagnetic events. Consequently, the satellite can be exposed to higher or lower levels than calculated with average models. Lozinski et al. [3] show that in the case of enhanced proton belts the solar panels can degrade by 5% before the satellite reaches its operational orbit. In space power budget equals money since it can determine the satellite lifetime.
Of course, in a competitive commercial environment, the GEO sector has also had to innovate to reduce costs, reducing development costs, reusing heritage “product line” units where possible and introducing more COTS hardware.
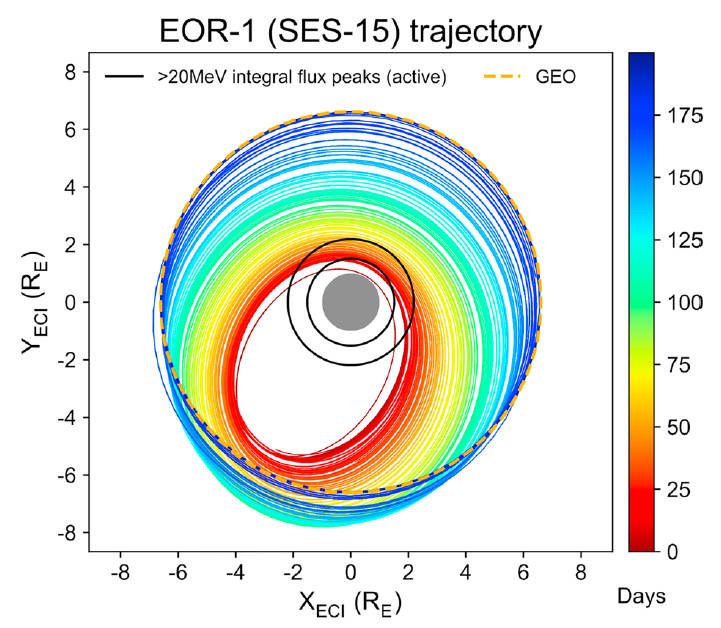
Figure 3: An electric orbit raising trajectory for the satellite SES-15. The colours refer to day after launch and show the slow change from a small red ellipse to the final blue circular GEO orbit. The black circles approximately delimit the proton belt. Credit: Lozinksi et al.
With these efforts to reduce costs might come some nagging concerns about reliability. An old saying goes: one man’s problem is another man’s opportunity and several concepts are maturing for orbital “tugs”. It’s not a new idea but maybe one whose time has come as it can combine advances in IT, propulsion and robotics (all need radiation hardness assurance of course). In some concepts the tugs could extend the life of GEO satellites which have run out of station-keeping fuel or lack electrical power. Active debris removal is one of the strongest motivations – capturing dead satellites and deorbiting them. Again it gets complicated - these space tugs themselves must be reliable; how will space tug services be funded? And of course the military will worry about whether a space tug is potentially an anti-satellite weapon.
For decades now universities and their offshoots have been working to make access to space affordable. These fantastic efforts have resulted in fast, capable missions (and ok several failures, to learn lessons by). We tend to think these days of CubeSats but let’s not forget the communities and efforts that already existed - like AMSAT and small satellite providers like SSTL/UoS. Their efforts have been picked up and applied more widely for applications and science, including new, flexible approaches to development – like “evolutionary” technology deployment and extensive use of carefully chosen COTS. The emergence of CubeSats has accelerated that process, although the rush into the concept led in the past to too many ill-prepared failures. Hopefully that phase is over and certainly there are many serious CubeSat developments under way. These projects do not ignore radiation issues but need to address them in affordable ways, including designing-in radiation tolerance. Heritage from tested hardware is of course important but what’s also interesting for RADNEXT are moves to irradiate complete spacecraft to screen for radiation effects, and generally access to low cost facilities for radiation testing is needed given typical very low project budgets.
So the space infrastructure in the coming decades will look radically different from the way it looks now. With all these new developments in commercial space, RADNEXT can expect to become a very important resource. RADNEXT includes a wide variety of facilities enabling work from low cost screening to investigation of highly specific issues, establishing new approaches but also allowing for traditional radiation effects investigations.
RADNEXT will also face some interesting problems where traditionally scientific facilities, familiar with collaboration models where results become public, work with commercial partners where this may not be desired. The radiation susceptibility of components, equipment or complete satellites measured in RADNEXT facilities by industry will be a commercially sensitive result. The problem becomes stickier when we work on strategic programmes such as European Galileo programme when radiation sensitivities will certainly not be public.
Super-sensitive Observatories
When it comes to science missions, the pressures are quite different. These can be huge investments both financially and in the efforts of large communities of scientists. Therefore, the approach to radiation hardness is much more targeted at minimising mission risk and maximising scientific return. The Athena X-ray astronomy observatory under development by ESA, to launch in 2031, builds on the great success of the XMM-Newton mission. It pushes the capabilities of the telescope and the detectors very hard. XMM was not without its radiation related problems and the detector and telescope teams had anticipated them. However, shortly before launch a new unanticipated phenomenon was discovered. The similar NASA mission Chandra was experiencing unexpected degradation of its detectors. The culprit was found to be low energy (<1MeV) “soft” protons scattering through the X-ray optics - a set of concentric shells that scatter incoming X-rays at low incidence angle to a focus on the detectors. Unfortunately, it was found that soft protons in the magnetosphere could do the same. Forewarned is forearmed, and careful use on XMM of a blocking filter has limited the damage. XMM is in an eccentric Earth orbit that results in some encounters with the radiation belts, causing unwanted radiation background resulting in instrument down-time. Since the time of XMM (and Integral and ISO - other missions in eccentric Earth orbits) it has become the norm to place astrophysics missions around the L2 Lagrange point. Here, apart from a milder radiation environment, spacecraft operations, thermal control and communications are simplified. Milder, but not completely eliminated. Payloads on ESA’s GAIA, Herschel and Planck missions at L2 saw mild but clear radiation effects [4-6] . Planck lost some 15% of bolometer data due to radiation background [5] . That’s not unusual. In space observing time equals money. On missions that often cost more than 1B€, loss of observing time clearly comes at a significant cost.
In his master’s thesis based on his work at ESAC, Christian Kirsch looked at the signals left by cosmic rays and solar energetic particles (SEP) on the GAIA CCDs [7] . Among many nice results, he shows how the CCD’s radiation interference follows the cosmic ray background but shows interesting features when solar particle events occur. While many events produce interference that corresponds to particle measurements made at L1 or geostationary orbit, others do not. This is a nice illustration of how SEP event fluxes can vary strongly in space as well as time. GAIA development had originally included a radiation monitor but unfortunately this was “descoped”. Its data would have been very useful.
Unsurprisingly Athena will also operate at L2. Its sensitivity will be fantastic, opening up more astrophysical frontiers. Sensitivity to X-rays of course means sensitivity to particle radiation and much work has been performed to minimise background. The quoted requirement for non-X-ray background in both main instruments is less than 5e-3 counts/cm2/s/keV. The extensive measures taken include: magnetic deflectors installed between the X-ray optics and the detectors [8] ; detailed graded shielding analysis and design (see e.g. Steffan Hauf’s Ph.D. Thesis [9] ); implementation of detector features such as anti-coincidencing to reject signals caused by charged particles; and processing of signals to remove background.
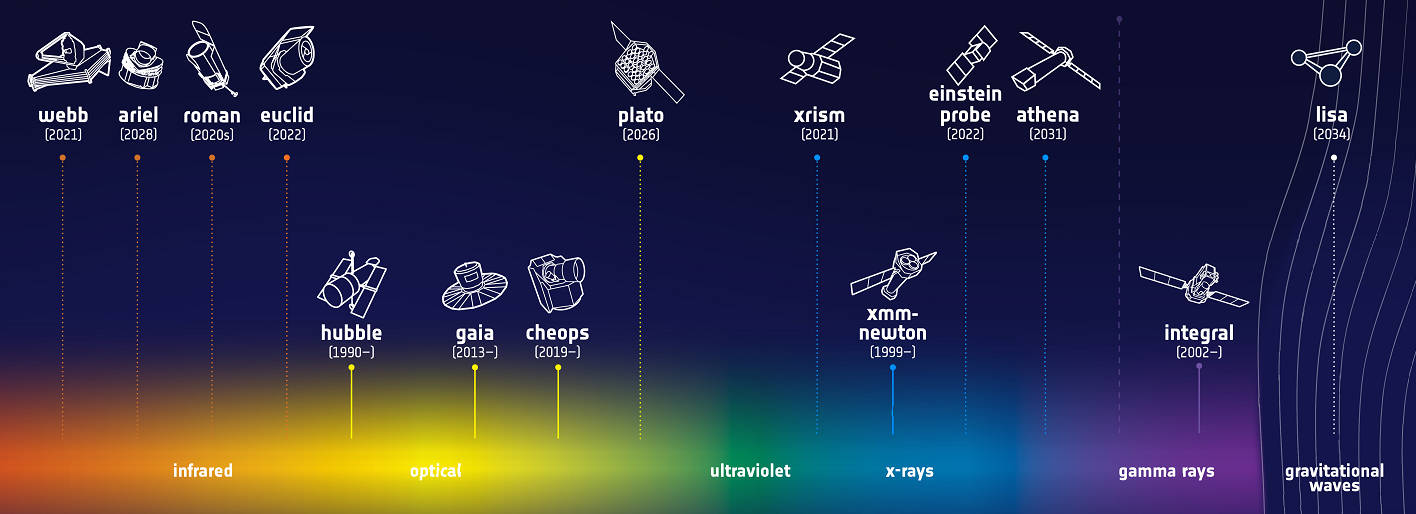
Figure 4: Currently operating and future ESA or collaborative astrophysics missions, Credit: ESA
Black Holes Merge
LISA takes the sensing of gravitational waves to new lengths, literally. The ground based and ground-breaking detectors of LIGO and Virgo detected gravitational waves created by merging compact black hole binaries, with frequencies in the range 10-1000Hz, whereas LISA will sense waves from massive black hole mergers and galactic binaries with much lower frequency (~mHz). LISA will be a triangular formation of 3 spacecraft 2.5e+6 km apart. As with the ground-based detectors the sensitivity required is astonishing - LISA will measure test-mass displacements of the order of a picometer and so reach a strain resolution of 1e-20 – amazing [10] .
And of course there is a sensitivity to radiation. It is one among many possible sources of disturbance to the “free falling” test-mass at the centre of the detectors. High energy particles can interact with the mass leaving a net charge that results in an electrostatic force. All the sources of disturbance are being intensively analysed and certainly the radiation issue is one of these [11] (I remember it being studied in ESA already in the 80’s). One interesting possibility being investigated is that the 3 LISA spacecraft carry radiation environment monitors. Being separated, they will not necessarily see the same environment, and a spin-off of the measurements would be interesting data on solar particle event spatial variations.
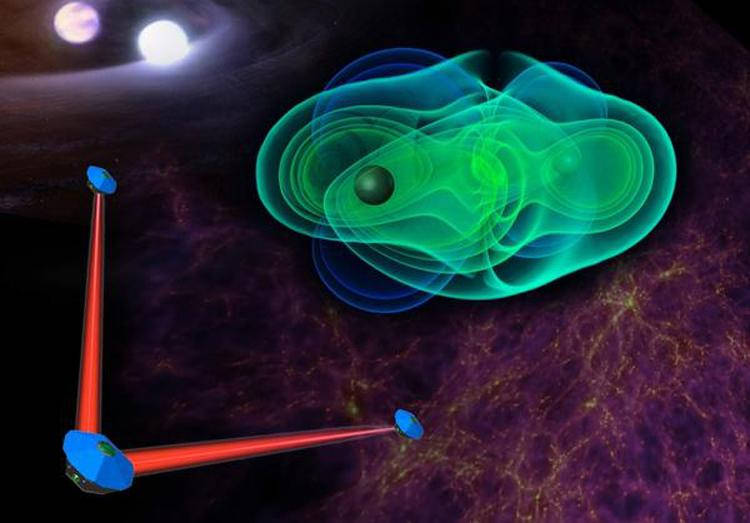
Figure 5: LISA mission, Credit: LISA Consortium
Human Spaceflight
Human spaceflight obviously has to take account of radiation. There are very well-established research lines to study radiation effects on humans, including for example the IBER projects [12] , many of whose collaborators are also in RADNEXT. It deserves a whole article of its own. but I’ll take the opportunity to make just a couple of points.
The first is that galactic cosmic rays, and resulting secondary radiations generated in vehicles or habitats, are the real problems for humans. Because solar events are more exciting/photogenic, we frequently read about the threats they pose to humans in space but in reality, apart from very extreme events, the real enemy is the high energy cosmic ray environment. Usually the high energy content of solar particle events is weak and they are easily shielded against, as are brief exposures to the radiation belts. But long term exposure to energetic cosmic ray ions leads to radiation health concerns.
The second point to flag is the knock-on problem of needing very high reliability in the life-critical hardware that supports human missions. Clearly such systems will be thoroughly evaluated for all radiation effects and here we do indeed need to be concerned about solar particle events and radiation belts and their variability. ESA and European industry are evolving the technology they developed for the 5 unmanned logistical transfer vehicles (ATVs) that re-supplied the ISS, to produce the European service modules (ESMs) for the Orion manned spacecraft. In addition, ESA will contribute elements of the Lunar Gateway itself, including ESPRIT modules for communications, viewing and refuelling, and contributions to the International Habitation Module. Unlike the LEO orbit of ISS, the near-lunar environment is fully exposed to interplanetary cosmic ay and solar energetic particle environments, so special care will be needed with ensuring the radiation hardness and safe functioning of the systems. Again, radiation test facilities will undoubtedly be important.
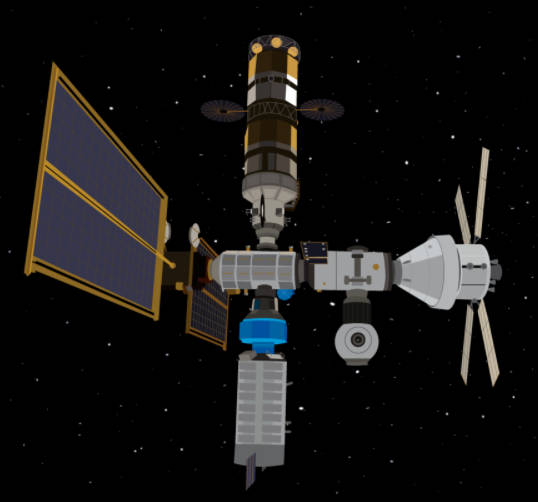
Figure 6: Lunar Gateway with on the extreme right Orion and its ESM, Credit: ESA
Final Remarks
The next 2 decades will see a dramatic and at times difficult transformation of the space domain, as exciting new science is done, humans move beyond LEO and the commercial sector grows enormously. I didn’t mention other sectors and Earth observation in particular is already experiencing enormous growth for monitoring the Earth and its environment for scientific and service reasons (e.g. ESA’s Earth Explorers, and the ESA, Eumetsat and EU Earth Watch, meteorological and Copernicus programmes). This growth has also been accompanied by growth in the complexities of payloads and on board processing.
The spin off for RADNEXT is that there will be a large demand to understand how many of these developments will be impacted by radiation effects. So RADNEXT partners can expect to be busy. RADNEXT brings a diversity to the problem which allows for various types of radiation investigations, ranging from screening to detailed experimentation. The facility types vary greatly including large laboratories, and smaller facilities and they will all address a need. But you can’t be sure when or even if they are needed. Or what is needed. Soft protons? Heavy ions? Energetic electrons? Neutrons? Mixed fields? Shielding experiments? Full detector susceptibility characterisation?
While a lot of the needs can be anticipated, experience shows that the unexpected will occur and then there is a rush to access the appropriate facility. RADNEXT makes that easier. On the other hand be wary of the manager or engineer who says we do not have a radiation issue on his/her mission/system/payload.